The two main types of ball valves—top entry and side entry—each offer distinct advantages depending on the specific requirements of the system. A top entry ball valve features a design where the valve’s ball is inserted from the top of the valve body, allowing for easier access during installation and maintenance. On the other hand, side entry ball valves have their ball inserted through the side of the valve body. This design tends to be more common in larger valve sizes and is typically used in systems where more robust construction and higher flow rates are required.
Top Entry Ball Valve: A Closer Look
What is a Top Entry Ball Valve?
A top entry ball valve is a type of ball valve where the ball, which controls fluid flow, is inserted into the valve body from the top. This design allows for easy access to the internal components, particularly when servicing or maintaining the valve. Unlike other valve types, which may require disassembly from the side or bottom, a top entry valve offers streamlined maintenance processes.
The key feature of a top entry ball valve is the ability to replace or service the ball, seals, and other internal components without fully removing the valve from the pipeline. This makes it a convenient choice for systems that require regular inspections or maintenance while minimizing downtime.
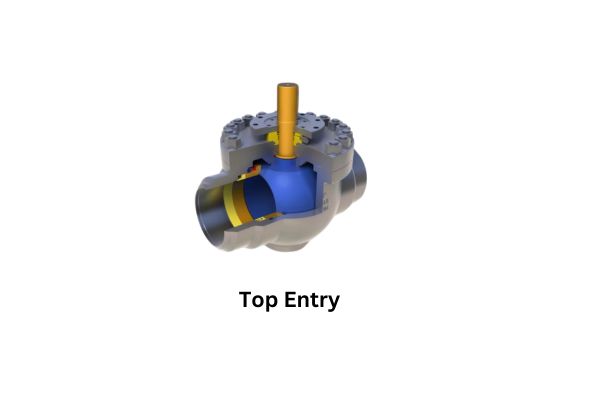
Key Design Features of a Top Entry Valve
Top entry ball valves have several distinct design elements that set them apart from side entry and other ball valve types:
Vertical Ball Insertion: The ball and its components are inserted from the top of the valve body, allowing for easy access and simplifying maintenance tasks like replacing seals or cleaning the valve.
Compact Design: The valve body is typically designed to be more compact than side entry valves, which makes it a good fit for spaces with limited room for large valve assemblies.
Simplified Maintenance: Since the valve ball and seat are accessible from the top, maintenance can be performed without the need to remove the entire valve body from the pipeline. This reduces the amount of time and effort needed for repairs or part replacements.
Ball Locking Mechanism: In some designs, the top entry valve features a locking mechanism to secure the ball inside the body, ensuring it stays in place during operation and preventing leaks.
Flow Path Control: The ball inside the valve rotates to align with ports, enabling the flow of fluid to be directed or shut off. The top entry design allows for precise control of the flow path while offering durability and high performance.
Advantages of Top Entry Ball Valves
Top entry ball valves offer several advantages that make them a preferred choice in various applications:
Ease of Maintenance: One of the primary benefits of top entry ball valves is the ease of maintenance. Since the valve’s internals are accessible from the top, maintenance can be done with minimal disruption to the piping system. This is especially useful in systems where frequent inspections and servicing are required.
Compact and Space-Saving: The compact design of a top entry valve allows it to be used in systems with limited space. It is ideal for tight installations or when working with large-scale systems where space optimization is critical.
Reduced Downtime: The ability to perform maintenance without removing the entire valve from the pipeline reduces system downtime. This makes top entry valves ideal for continuous operations where efficiency and uptime are essential.
Ideal Applications for Top Entry Ball Valves
Top entry ball valves are used across a variety of industries, particularly in applications that demand easy access for maintenance and operation efficiency. Some of the most common applications include:
Oil and Gas Industry: Top entry ball valves are widely used in the oil and gas sector, where their ability to withstand high pressures and corrosive environments is highly valued. Their easy maintenance and durability make them ideal for upstream, midstream, and downstream applications.
Chemical Processing: In chemical processing plants, top entry ball valves are ideal for handling aggressive chemicals and fluids under high pressure. The quick access to internal components allows for regular maintenance and ensures the safe handling of hazardous materials.
Water Treatment Facilities: These valves are commonly used in water treatment systems, where frequent inspections and cleaning of valves are necessary. The ability to access the valve internals from the top makes servicing these valves easier and faster, minimizing downtime.
Side Entry Ball Valve: A Closer Look
What is a Side Entry Ball Valve?
A side entry ball valve is a type of ball valve where the ball is inserted into the valve body from the side, rather than the top. This design is typically used for larger valve sizes and more robust systems where higher flow rates or more substantial construction are necessary. Unlike top entry ball valves, which offer easy access for maintenance and servicing, side entry valves are often more durable and provide superior sealing capabilities under high-pressure and high-temperature conditions.
The ball inside a side entry valve rotates to control fluid flow, either by opening, closing, or diverting it to multiple outlets, depending on the valve configuration. Side entry valves are designed to handle more demanding operational environments and are known for their reliability and long-lasting performance in various industrial settings.
Key Design Features of a Side Entry Valve
Side entry ball valves come with distinct design characteristics that set them apart from other valve types. Here are some of the key features:
Side Insertion of the Ball: The most noticeable feature is the side insertion of the ball into the valve body. This design requires disassembly from the valve’s side to access the internal components, which can make servicing more complex compared to top entry valves.
Heavy-Duty Construction: Side entry valves are typically made with heavier materials to withstand the demands of high-pressure or high-temperature applications. They are designed for long-term use in industrial systems and are built to endure harsh operational conditions.
Ball and Stem Mechanism: The ball inside the side entry valve is rotated by the stem, which is connected to the actuator or manual handle. The mechanism ensures smooth and efficient operation, offering precise flow control.
Durable Seals and Seats: Side entry valves are designed with robust seals and seats, which help ensure leak-tight performance even under extreme pressure or temperature. The valve seats are often made from materials like PTFE or metal alloys to provide excellent sealing.
Large Valve Sizes: Side entry ball valves are often used in larger pipeline systems due to their robust construction, which can support higher flow rates and heavier operational demands. These valves are typically found in larger industrial applications.
Multiple Port Configurations: Similar to top entry ball valves, side entry valves can also be designed with multiple ports for diversion, mixing, or isolation of fluids. This flexibility allows for precise fluid flow management in complex systems.
Advantages of Side Entry Ball Valves
Side entry ball valves offer several distinct advantages that make them suitable for heavy-duty applications:
Higher Pressure and Temperature Handling: Due to their construction and materials, side entry valves are better suited for high-pressure and high-temperature applications compared to top entry valves. They provide robust sealing and durable operation in demanding environments, making them ideal for industries like oil and gas, chemical processing, and power generation.
Better Performance in Larger Systems: Side entry valves are often used in larger pipeline systems, where high flow rates are required. They can handle larger volumes of fluid without compromising on performance, making them ideal for industrial applications that need heavy-duty solutions.
More Robust Sealing: The seals in side entry valves are designed to withstand higher stresses, and the valve’s body structure is reinforced for leak-tight performance, even under difficult conditions. This makes side entry ball valves especially reliable in systems where the prevention of leaks is critical.
Ideal Applications for Side Entry Ball Valves
Side entry ball valves are designed for applications where high flow rates, high pressure, and reliable sealing are essential. Their robust construction makes them ideal for industries and systems that need heavy-duty valves capable of withstanding extreme conditions. Here are some of the ideal applications:
Oil and Gas Industry: Side entry ball valves are commonly used in the oil and gas industry, particularly in upstream operations, to control the flow of fluids under high pressures. These valves provide excellent sealing and handling of corrosive and abrasive fluids, making them essential for pipeline systems and offshore rigs.
Chemical Processing: In chemical processing plants, side entry valves are ideal for controlling the flow of aggressive chemicals and gases. The heavy-duty construction and superior sealing capabilities make them perfect for handling volatile substances and operating in extreme temperature environments.
Power Plants: Side entry ball valves are used in power plants for controlling steam, water, and fuel flow in turbines, boilers, and heat exchangers. Their ability to handle high-pressure systems makes them an essential part of energy production and distribution.
Top Entry vs Side Entry Ball Valve: Key Differences
Structural and Functional Differences
The structural and functional differences between top entry and side entry ball valves primarily revolve around the method of ball insertion, body construction, and how these factors affect overall performance.
Ball Insertion:
Top Entry Ball Valve: The ball is inserted from the top of the valve body, allowing for easy access to internal components during maintenance or replacement. This design often results in a more compact valve body and simplified access for servicing.
Side Entry Ball Valve: In contrast, the ball in a side entry valve is inserted through the side of the valve body. This requires more complex disassembly during maintenance, but it allows for a stronger, more durable construction, particularly for larger valves.
Valve Body Construction:
Top Entry Ball Valve: Typically features a smaller, more compact body design, often used in smaller systems or installations where space constraints are a factor. The body structure is generally simpler and easier to manufacture.
Side Entry Ball Valve: These valves are designed with a more robust body, particularly for handling high pressures and large flow rates. Side entry ball valves are usually larger, making them better suited for heavy-duty industrial applications.
Flow Path and Pressure Handling:
Top Entry Ball Valve: These valves generally handle lower to moderate pressures and flows, as their design is more suited for smaller pipelines or systems with less demanding operational requirements.
Side Entry Ball Valve: Side entry valves, being sturdier and larger in design, are ideal for high-pressure and high-flow systems, often found in industries like oil and gas, power generation, and chemical processing.
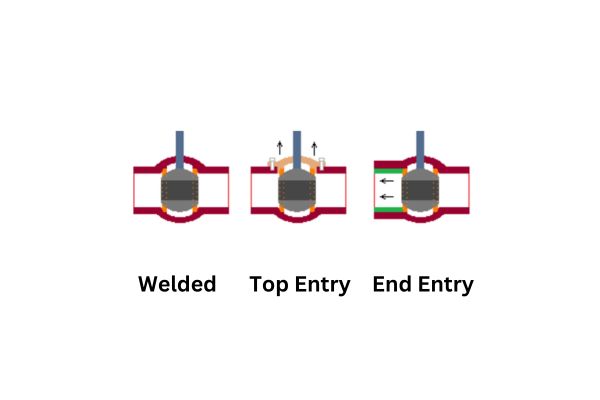
How Valve Operation Differs Between the Two
The operation of both top entry and side entry ball valves is largely the same, relying on a rotating ball to control fluid flow, but the difference in their design influences certain operational aspects.
Ease of Actuation:
Top Entry Ball Valve: Since the ball is inserted from the top, these valves typically have a more straightforward mechanism for actuation, often requiring less space for turning the ball. This makes them suitable for automated or manual control in compact systems.
Side Entry Ball Valve: The side entry design can sometimes make the actuation mechanism slightly more complex, especially in larger valves where the ball size and sealing requirements increase. However, the overall function remains consistent with that of the other ball valves—quarter-turn operation to start, stop, or divert flow.
Rotation and Flow Control:
Both valve types allow quarter-turn operation to control flow, but due to the structural design, side entry ball valves may be more effective at handling larger volumes of fluid. The rotating ball in both types ensures flow control through different ports, either for diverting, mixing, or isolating flow, but side entry valves are typically favored for systems requiring more durable and robust construction for large-scale flow management.
Comparing Installation and Maintenance
Installation and maintenance are key factors when choosing between top entry and side entry ball valves. Here’s how they compare:
Installation:
Top Entry Ball Valve: Installation of top entry valves tends to be simpler due to their compact design and easier access to valve internals. They can be more easily adapted for smaller piping systems where space and accessibility are limited.
Side Entry Ball Valve: Installing side entry valves can be more complex, especially in larger systems where handling large valve sizes is required. These valves need to be properly aligned and may require more space for installation due to their size and robust construction.
Maintenance:
Top Entry Ball Valve: The main advantage of top entry ball valves when it comes to maintenance is that they allow for easy access to the internal components by removing the valve top. This is ideal for applications requiring frequent inspections, as the valve internals, including the ball and seals, can be serviced without having to remove the entire valve from the system.
Side Entry Ball Valve: Maintenance of side entry valves can be more challenging. Since the ball is inserted from the side, full disassembly is often required for internal repairs. This can lead to longer downtime and higher maintenance costs, especially in systems where valve accessibility is limited.
Durability and Service Life:
Top Entry Ball Valve: While top entry valves offer ease of maintenance, their construction is typically more suited for moderate-duty applications. They may not last as long under high pressure or in environments with harsh chemicals.
Side Entry Ball Valve: Side entry valves are generally built for heavy-duty applications, which means they are likely to have a longer service life in demanding systems. Their robust design allows them to handle extreme pressures and fluid types, ensuring durability over time.
Flow Control and Pressure Handling Differences
Flow control and pressure handling are two of the most significant factors in determining which type of valve is suitable for a particular application.
Flow Control:
Top Entry Ball Valve: Top entry valves are commonly used in smaller pipeline systems where precise control of fluid flow is required but the volume and pressure of the fluid are not extreme. They are excellent for situations where flow direction needs to be changed, but the scale of the operation is more compact.
Side Entry Ball Valve: Side entry valves excel in high-flow applications, where the volume of fluid passing through the valve is considerable. They are typically used in industrial-scale systems, such as oil pipelines, large-scale chemical processing, and power generation, where the ability to handle large amounts of fluid without loss of pressure is paramount.
Pressure Handling:
Top Entry Ball Valve: These valves can handle moderate pressure systems, making them ideal for municipal water systems, residential plumbing, and certain chemical applications where the pressures involved are not extreme.
Side Entry Ball Valve: Side entry ball valves are specifically designed for high-pressure environments. They are better equipped to handle severe operating conditions, such as those found in oil rigs, refineries, and large power plants, where pressure needs to be maintained over long periods.
Valve Sealing and Performance:
Top Entry Ball Valve: Top entry valves generally perform well under normal operational conditions but may experience wear and tear more quickly than side entry valves when used in high-pressure or abrasive environments.
Side Entry Ball Valve: Side entry valves provide superior sealing capabilities and are ideal for high-pressure systems. Their design ensures that the ball and seals remain securely in place, even under extreme stress, resulting in better overall valve performance in demanding applications.
Conclusion
Selecting the right valve depends on factors such as system size, pressure, flow requirements and maintenance frequency to ensure efficiency and reliability. If you need any kind of hydraulic ball valves, please feel free to contact us!
FAQ
What is a top entry ball valve?
A top entry ball valve is a valve in which the ball is inserted through the top of the valve body and is designed for easy servicing and replacement of internal components in systems where space is limited.
What is a side entry ball valve?
A side entry ball valve is a valve where the ball is inserted from the side of the body, typically used for larger valves and high pressure, high flow industrial systems.
What is the main difference between top entry and side entry ball valves?
The top entry ball valve has a top entry design for ease of maintenance and installation, while the side entry ball valve is inserted through the side for a more robust construction suitable for high pressures and high flow rates.
What applications are top entry ball valves suitable for?
Top entry ball valves are suitable for smaller systems, low to medium pressure requirements, and frequent maintenance environments.
What applications are side entry ball valves used for?
Side entry ball valves are used in large, high-pressure, high-flow industrial systems, to withstand extreme operating conditions.
What are the maintenance differences between top entry and side entry ball valves?
The top entry ball valve is easier to maintain because the internal components can be accessed and replaced directly from the top, whereas the side entry ball valve needs to be disassembled from the side, which is more complicated to maintain, but is more suited to industrial systems that operate steadily for long periods of time.